Evaporation and Intermolecular Attractions
- Phoo Pwint Thaung Sein
- Nov 17, 2024
- 12 min read
Updated: Nov 20, 2024
Phoo Pwint Thaung Sein
Abstract
The experiment aims to find the effect of the intermolecular forces and molar mass of chemicals on the change in temperature during evaporation by the endothermic process, measured using temperature probe and logger pro. The research question is how does the strength of intermolecular attractions, influenced by the type of forces and molar mass of substances, affect the change in temperature during evaporation processes? As the experiment unfolds, the focus lies on exploring the molecular mass and structure of organic compounds, seeking to understand the presence and relative strength of three fundamental intermolecular forces: hydrogen bonding, dipole-dipole forces, and London dispersion forces. The process of evaporation is a dynamic molecular phenomenon, wherein molecules at the liquid surface acquire the necessary energy to overcome intermolecular forces and transition into the gas phase. This energy absorption from the surroundings results in a cooling effect, a characteristic feature of endothermic processes (CK12-Foundation). The Temperature Probe, coupled with Logger Pro, will be used as a precise tool to measure the temperature changes associated with the evaporation process. The unique molecular mass and structure of each compound contribute to variations in the strength of these forces, influencing the observed temperature differences. This exploration extends beyond the immediate objectives, offering a nuanced understanding of the molecular dynamics that govern evaporation. The experiment not only advances our knowledge of fundamental chemical processes but also sets the stage for comprehending the broader implications of intermolecular forces in diverse scientific contexts, from materials science to environmental studies.
Introduction
Van der Waals forces are essential intermolecular attractions that influence how molecules interact. These forces consist of three main components: London Dispersion Forces, Dipole-Induced Dipole Forces and Dipole-Dipole Forces. London Dispersion Forces, often called van der Waals forces, arise from momentary fluctuations in electron distribution within molecules, creating temporary dipoles that induce similar dipoles in neighboring molecules. These forces are more likely to be found in larger molecules due to increased electron cloud size. Dipole-Dipole Forces, on the other hand, occur between polar molecules with permanent dipole moments, resulting from differences in electronegativity between bonded atoms. Hydrogen bonding is a specific and strong type of dipole-dipole interaction. It occurs when a hydrogen atom is covalently bonded to a highly electronegative atom like oxygen, nitrogen, or fluorine. The hydrogen atom forms a strong electrostatic interaction with the electronegative atom in another molecule, creating a distinctive and potent force. Thus, hydrogen bonds are generally stronger than van der Waals forces. This strength arises from the significant electronegativity difference between hydrogen and the electronegative atom, leading to a robust electrostatic attraction. (BROWN et al. 960)
In addition, if the compounds have the same intermolecular forces, the molar mass of substances becomes one of the significant things in determining their properties. Larger molecules, characterized by higher molar masses, exhibit increased strength of intermolecular forces due to their larger number of electrons and atoms. These substances often require more energy to transition from a liquid to a gas phase during processes like evaporation. The relationship between the strength of intermolecular attractions, influenced by both the type of forces and the molar mass, and the volatility of a substance is governed by the principles of thermodynamics. Substances with stronger intermolecular attractions, such as those with hydrogen bonds or higher molar masses, demand more energy to overcome these forces during phase transitions. This requirement results in lower volatility, indicating a reduced ability to evaporate. Conversely, substances with weaker intermolecular attractions have lower energy requirements for vaporization, enabling molecules to more easily overcome the forces holding them in the liquid phase, leading to higher volatility. (BROWN et al. 960)
If the strength of intermolecular attractions increases, as determined by the type of forces and molar mass of substances increases, then the change in temperature, measured by their ability to evaporate during processes like evaporation, will decrease (BROWN et al. 960). This hypothesis is based on the understanding that stronger intermolecular forces, such as hydrogen bonding, and larger molar masses demand more energy to overcome during phase transitions, leading to reduced volatility or an ability to evaporate. On the other hand, substances with weaker intermolecular forces, characteristic of London Dispersion Forces, and lower molar masses are expected to have lower energy requirements for vaporization, resulting in higher volatility.
Chemical | Formula | Structural Formula | Molecular weight (g/mol) | Intermolecular Force |
Acetone | (CH3)2CO | ![]() | 58.08 | Dipole- dipole (C=O) London Dispersion |
Cyclohexane | C6H12 | ![]() | 84.16 | London Dispersion |
Methanol | CH3OH | ![]() | 32.04 | Hydrogen Bond (O-H) Dipole-Dipole London Dispersion |
Ethanol | C2H5OH | ![]() | 46.068 | Hydrogen Bond (O-H) Dipole-Dipole London Dispersion |
Propan-1-ol | C3H7OH | ![]() | 60.0952 | Hydrogen Bond (O-H) Dipole-Dipole London Dispersion |
Butan-1-ol | C4H9OH | ![]() | 74.12 | Hydrogen Bond (O-H) Dipole-Dipole London Dispersion |
(Table 1) Structural Formula, Molecular weight and Intermolecular Force of Chemicals
(Wade), (PubChem, “Acetone”), (PubChem, “Cyclohexane”)
Controlled variable (CV)
Variable | Method of control | Reasons of control | Specification |
1) Amount of chemical compounds | Volume of controlled using a 7 ± 0.05cm height filled | The height of the probe touching with the chemical will be the same only when the same amount of chemical is put in the same test tubes. | 1) 7 ± 0.05cm |
2) Time (Initial & final temperature) | Start recording only when the temperature reaches to equilibrium state (stable temperature shown for 30 seconds while dipping the probe in the chemical. Final temperature is recorded only when the temperature goes up again (after taking off from the test tube). Then, take the minimum point. | Starting recording before equilibrium or missing the minimum temperature point could result in inaccurate temperature change measurements and comparison. | 2) Equilibrium stage |
3) Size of the filter paper | Cut the same size filter papers 2.5 x 2.5 cm | Varied filter paper sizes could introduce an additional factor influencing the rate of evaporation, confounding the impact of intermolecular forces and molar mass. | 3) 2.5 x 2.5 cm |
4) Room Temperature | Make the same room condition and temperature in the whole experiment. | Fluctuations in room temperature could introduce variations in the rate of evaporation, complicating the interpretation of temperature changes. | 4) 20-25°C |
5) Initial temperature of probe & liquid | Clean the probe with distilled water after every trial and increment. Clean the test tubes, pipette and funnels with distilled water. Use different pipettes and funnels for different chemicals. | Residual impurities could change the chemical properties, leading to unpredictable temperature changes and undermining the precision of the experiment. | 5) 20-25°C |
6) Size of the test tubes | The same size test tubes are used in experiments to ensure consistency in volume of liquid used. | Varied test tube sizes could introduce volume-dependent differences, making it challenging to attribute temperature changes solely to intermolecular forces and molar mass. | 7) Same size for all trials |
7) The placement of the temperature probe | Touching the test tube (glass) can affect the observed temperature because glass has a different ability to hold heat compared to other materials. Additionally, the way the probe is positioned after being taken out from the test tube should be consistent. | Different environmental conditions can influence how much the temperature changes, and keeping the probe placement the same helps maintain reliability in our observations. | 8) 5cm over the edge of the table |
(Table 2) Controlled Variables
Experimental Setup
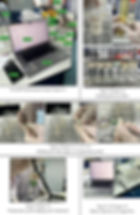
Methods
Test tubes were filled with organic compounds to a height of 7 cm, and each was labeled with the corresponding compound name. The test tubes were then cleaned with distilled water to ensure the removal of any impurities. Different pipettes and funnels were used to transfer the compounds into the test tubes, which were subsequently placed in a test tube rack. The Logger Pro software was opened, and the "09 Evaporation" file from the Chemistry with Vernier folder was accessed. A temperature probe was connected to the software. Filter papers were cut into six pieces, each measuring 2.5 x 2.5 cm. The temperature probe was wrapped with one of the cut filter papers and secured with a rubber band to ensure stability. The paper was positioned evenly with the probe's end. The wrapped temperature probe was then placed into the test tube containing acetone, and pieces of tape were used to stabilize the probe's position. The probe was left in the acetone until it reached equilibrium, which took at least 30 seconds. The initial temperature of the acetone was recorded over 15 seconds by clicking "collect" in Logger Pro. Once the initial temperature was established, the probe was simultaneously removed from the acetone test tube, and its tip was taped to extend approximately 5 cm over the edge of the table. The temperature change was monitored until it reached its minimum and began to rise. Data collection was stopped when the temperature started to increase, and both the maximum (initial temperature) and minimum (final temperature) values for acetone were recorded. The temperature probe was then cleaned with distilled water and allowed to return to normal temperature before repeating the procedure. This process was repeated three more times to obtain four trials for acetone. The same steps were followed for five other compounds: cyclohexane, methanol, ethanol, propan-1-ol, and butan-1-ol.
Data Processing
Process number | Formula |
Process 1 | Formula: Average = Total amount/Numbers of trial ![]() Example: Average temperature difference = 16.50+19.80+15.30+19.004 = 17.60 (roundup 2 decimals) ![]() |
Process 2 | Formula: (Maximum-Minimum)Value/2 ![]() Example: Absolute error of Avg Temperature difference in acetone = (19.80-15.30)/2 = 2.30 |
Process 3 | Formula: Absolute error/Average Value x 100 ![]() Example: Error bar percentage of time = 2.26/17.65 x 100 = 12.81 % |
Safety Considerations
Safety Categories | Description | How this can be avoided |
Physical | A. The broken glasses can cause injuries. B. Laptops are sensitive to any liquids. C. If a person accidentally gets chemicals in the sensitive areas (eye, nose, mouth, etc…) it causes discomfort, redness, irritation and even serious conditions. | A. Be careful when holding the materials with glass. B. Don’t put unnecessary devices on the table. Put the laptops away from the chemicals. C. Wear the goggles, coats and gloves in the experiment. |
Environmental Concerns | A. Although other compounds can be poured in the basin, cyclohexane poses a significant environmental risk due to its toxicity and potential to contaminate ecosystems. Improper disposal of this chemical can result in soil and water pollution, impacting aquatic life and ecosystems while contributing to air pollution. Additionally, its persistence and ability to bioaccumulate in living organisms heighten its environmental impact. | A. To mitigate these risks, reusing cyclohexane rather than disposing of it is a responsible choice. This approach aligns with environmental regulations, minimizing the amount released into the environment, thereby reducing the potential harm caused by this hazardous compound and supporting a more sustainable chemical management practice. (PubChem, “Cyclohexane”) |
(Table 3) Safety Considerations
Data
Qualitative Data
How does the temperature and physical appearance change in different types of compounds? |
All compounds remain colorless, clear and not sticky. The temperature is higher in the beginning of the process and lower over time (after taking out from the test tube). |
Quantitative Data
Raw Data Table - Chemical vs. Temperature
Trial 1 | Trial 1 | Trial 2 | Trial 2 | Trial 3 | Trial 3 | Trial 4 | Trial 4 | |
Chemical Name | Initial Temp | Final Temp | Initial Temp | Final Temp | Initial Temp | Final Temp | Initial Temp | Final Temp |
Acetone | 26.90 | 10.40 | 26.50 | 6.70 | 24.77 | 9.49 | 26.10 | 7.10 |
Cyclohexane | 27.80 | 10.30 | 24.30 | 10.90 | 24.29 | 10.93 | 25.80 | 11.10 |
Methanol | 26.70 | 11.60 | 28.20 | 9.20 | 24.09 | 9.10 | 26.40 | 9.10 |
Ethanol | 27.30 | 15.30 | 27.30 | 15.10 | 26.63 | 14.16 | 26.40 | 14.90 |
Propan-1-ol | 26.60 | 20.00 | 27.10 | 19.00 | 25.10 | 17.88 | 27.10 | 18.80 |
Butan-1-ol | 27.50 | 21.80 | 27.10 | 22.70 | 25.27 | 21.18 | 27.10 | 21.00 |
(Table 4.1) The collected data of initial and final temperatures in 5 trials in each of 6 different chemicals
Processed Data Table
Chemical Name | Trial 1 | Trial 2 | Trial 3 | Trial 4 | |||
Acetone | 16.50 | 19.80 | 15.28 | 19.00 | 17.65 | 2.26 | 12.81 |
Cyclohexane | 17.50 | 13.40 | 13.36 | 14.70 | 14.74 | 2.07 | 14.05 |
Methanol | 15.10 | 19.00 | 14.99 | 17.30 | 16.60 | 2.00 | 12.07 |
Ethanol | 12.00 | 12.20 | 12.46 | 11.50 | 12.04 | 0.48 | 3.99 |
Propan-1-ol | 6.60 | 8.10 | 7.23 | 8.30 | 7.56 | 0.85 | 11.25 |
Butan-1-ol | 5.70 | 4.40 | 4.09 | 6.10 | 5.07 | 1.01 | 19.86 |
According to the graph and qualitative data table, in alcohols with the same functional group (-OH) and hydrogen bonding, a clear trend emerges: as molar mass decreases, the temperature difference during evaporation increases. This is exemplified by methanol, with a lower molar mass of 32.04 g/mol, exhibiting the highest temperature difference among alcohols. Inversely, butan-1-ol, with a higher molar mass of 74.12 g/mol, shows the lowest change in temperature. This trend shows the influence of molecular weight in the same intermolecular forces occurring compounds on evaporation behavior. The bigger molar mass, the stronger the bond is and less evaporation which means less temperature change. Examining compounds with varied structures and bonding (acetone, cyclohexane vs ethanol, propan-1-ol, butan-1-ol) reveals a general trend where stronger intermolecular forces lead to lower temperature differences. Since acetone only has dipole-dipole, it has a bigger temperature difference than propan-1-ol and butan-1-ol, compounds containing the strongest intermolecular force: hydrogen bonding. This aligns with expectations, as compounds with stronger intermolecular forces require more energy input for the phase transition from liquid to gas. An exception arises when comparing cyclohexane, a nonpolar compound with only London dispersion forces, to acetone and methanol. Despite its non-polar nature, cyclohexane, with a significantly higher molar mass, exhibits a lower temperature difference than acetone and methanol, suggesting that compound strength involves factors beyond intermolecular forces.
Throughout the experiment, the endothermic nature of the evaporation process becomes evident. As compounds transition from liquid to gas, they absorb heat energy from their surroundings, resulting in a cooling effect. This cooling effect is biggest in compounds with weaker intermolecular forces and lower molar masses, as they tend to evaporate more readily (BROWN et al. 960). In conclusion, the experiment not only reveals nuanced relationships between compound properties and evaporation behavior but also emphasizes the multifaceted nature of the endothermic process. By considering factors such as molar mass and intermolecular forces, this exploration contributes to a well understanding of thermodynamics in chemical processes, making a foundation for further investigations into the complexity of endothermic reactions.
Evaluation:
Our hypothesis of higher intermolecular forces and molar mass would take more energy to break the bonds resulting in the smaller temperature change is supported with the results and collected data. While the experiment generally followed the correct scientific methods, several aspects could have been improved to enhance the accuracy and reliability of the results. One issue was the inconsistency in recording the initial temperature before the system had fully reached equilibrium. If the temperature was recorded prematurely, this led to a larger-than-accurate observed temperature change, potentially skewing the data. Ensuring that the probe was left in the test tube until the temperature remained stable for at least 10 seconds would have provided more precise measurements. Another significant challenge was the positioning of the temperature probe within the test tube. If the probe touched the glass surface, the recorded temperature could have been influenced by the glass's specific heat capacity rather than the liquid, introducing variability in the results. Inconsistent probe placement across trials may have also contributed to discrepancies in the data. Standardizing the position of the probe in each trial and ensuring it did not contact the glass would have minimized these errors. Time constraints during data collection posed another limitation. The initial 180-second observation period may not have been long enough to capture the complete endothermic process, potentially leading to incomplete data. This limitation is particularly significant as it may have resulted in the need for additional trials, consuming more time and resources. Extending the observation time to 500 seconds in future experiments would help to ensure that the entire process is captured, leading to more accurate results. Lastly, while most of the data was consistent, one data point had a significantly higher error percentage of 19.9%, indicating potential inconsistencies in the experimental setup or execution during that trial. Addressing these issues through more rigorous control of variables and adherence to standardized procedures would reduce such errors in future experiments.
Discussion
Our hypothesis that substances with higher intermolecular forces and greater molar mass would require more energy to break their bonds, resulting in smaller temperature changes during evaporation, was supported by the experimental results. The data showed a clear correlation between these factors, reinforcing the understanding of how molecular interactions govern the physical behavior of substances.
This experiment provided valuable insights into the relationship between intermolecular forces, molar mass, and evaporation behavior. Such knowledge is crucial in various real-world applications, particularly in the pharmaceutical industry and environmental science. In pharmaceuticals, understanding these relationships is vital for designing drug formulations with optimal bioavailability and therapeutic efficacy. For instance, compounds with stronger intermolecular forces may exhibit lower volatility, which can influence the rate of drug release and absorption in the body. This insight helps in the development of drug formulations that achieve the desired therapeutic effect while minimizing side effects.
In environmental science, the evaporation characteristics of solvents play a significant role in determining their impact on air quality and pollution. Substances with weaker intermolecular forces and lower molar masses tend to have higher volatility, leading to faster evaporation and greater atmospheric emissions. By understanding these properties, researchers and industry professionals can select solvents that are less harmful to the environment, contributing to cleaner and greener industrial practices.
However, while the experiment reinforced known principles, it also highlighted areas where further research is needed. One gap in knowledge is the extent to which these findings apply to more complex mixtures of substances, where multiple types of intermolecular forces might be at play simultaneously. Additionally, the influence of environmental factors such as temperature, pressure, and humidity on the evaporation behavior of substances with varying intermolecular forces and molar masses warrants further investigation. Addressing these gaps would enhance our ability to predict and manipulate the behavior of chemical compounds in real-world applications, leading to more efficient and sustainable practices across various industries.
In summary, the experiment not only confirmed our hypothesis but also underscored the broader relevance of the findings. The results contribute to a deeper understanding of the interplay between molecular structure and physical behavior, with significant implications for fields ranging from pharmaceuticals to environmental science. Nonetheless, further research is needed to fully explore the complexities of these relationships and to apply this knowledge in more diverse and practical contexts.
Works Cited
BROWN, CATRIN, et al. Pearson Baccalaureate: Chemistry for the IB Diploma Programme Higher Level, 3rd Edition. 2023. 3rd Edition, Pearson Education Limited, 2023, p. 960.
CK12-Foundation. Flexbooks.ck12.org, 27 Feb. 2022, flexbooks.ck12.org/cbook/ck-12-chemistry-flexbook-2.0/section/17.4/primary/lesson/endothermic-reactions-ms-ps/.
PubChem. “Acetone.” National Library of Medicine, 2019, pubchem.ncbi.nlm.nih.gov/compound/acetone.
PubChem. “Cyclohexane.” National Library of Medicine, 2019, pubchem.ncbi.nlm.nih.gov/compound/cyclohexane
Wade, Leroy G. “Alcohol - Physical Properties of Alcohols.” Encyclopædia Britannica, 28 Feb. 2019, www.britannica.com/science/alcohol/Physical-properties-of-alcohols.
All Photo credits to Author, Thaung Sein.